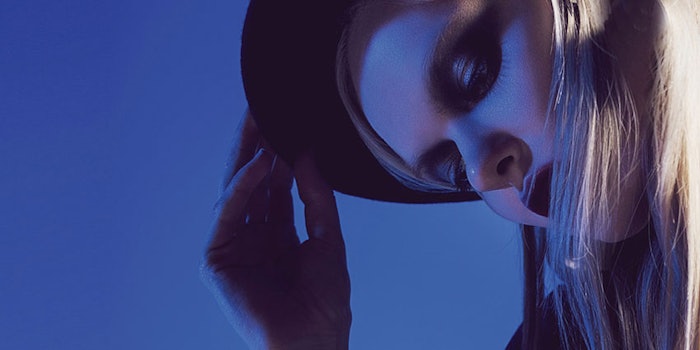
As you peruse the content of Cosmetics & Toiletries, you will see we live in a world that caters very highly to the sense of vision. Thanks to their intricate biology, our eyes can detect light and transmit not only information about the image we are seeing, but also how vivid the colors are within that image and how intensely they appear. Indeed, at times it seems as though our vision allows us to see a near infinite range of colors.
Log in to view the full article
As you peruse the content of Cosmetics & Toiletries, you will see we live in a world that caters very highly to the sense of vision. Thanks to their intricate biology, our eyes can detect light and transmit not only information about the image we are seeing, but also how vivid the colors are within that image and how intensely they appear. Indeed, at times it seems as though our vision allows us to see a near infinite range of colors.
However, the reality is our vision only allows us to see a small portion of the light spectrum—aptly named the visible spectrum, which ranges in wavelength from approx. 400–700 nm.
We interact with light in the visible spectrum on a daily basis. For most of us, vision is a part of our daily routine, so it is easy to forget the visible light around us is interacting with everything it illuminates, including our skin. Just like UV light, visible light can penetrate the skin. And while UVB can penetrate through the epidermis to the upper dermis, and UVA can penetrate further through the dermal layer, visible light can pass through both the epidermal and dermal layers to reach the subcutaneous adipose tissues—and perhaps even deeper.1
Since the adverse and dangerous effects of UV radiation on skin biology are well-known, this raises an important question: If this seemingly benign visible light can penetrate the skin, does it also have a biological impact? The answer is a definite yes. In fact, for years, visible light therapy has been successfully used in the medical field to treat skin disorders such as acne and chronic inflammatory conditions associated with hyperproliferating skin cells.2
But what about outside of medical application—can visible light still impact skin on a daily basis? The answer, again, is a definite yes. While the intensity of light we are exposed to on a daily basis is nowhere near the intensity of light used in the medical field for therapeutic purposes, research suggests that daily exposure to visible light can nonetheless promote damage to the skin, and that this damage may be more associated with specific wavelengths (colors) of visible light than others.
Biological Effects of Visible Light in Skin
Visible light has long been known to play a role in certain types of photodermatoses, especially solar urticaria, chronic actinic dermatitis and cutaneous porphyrias.3 While these extreme pathological responses are not common, visible light also provokes reactions in normal skin. For example, in a study using a human epidermal tissue equivalent model, when the tissue was irradiated with visible light (400–700 nm), a dose-dependent increase in the formation of reactive oxygen species (ROS) was observed, as was an increase in the release of both IL-1α and MMP-1.4 These responses are very similar to those observed in the skin irradiated with UV light.
This raises another question—are the effects observed in this study due to the entire spectrum of visible light used, or are discrete portions of the spectrum responsible?
The Dangers of Blue Light
It is now recognized that non-UV-containing light particularly in the violet-blue portion of the visible spectrum (400–500 nm) may be responsible for most of the adverse effects caused by the entirety of visible light. This portion of the visible spectrum has been termed High Energy Visible Light (HEV), as it is the most energetic. Although it technically includes the blue, indigo and violet portions of the visible spectrum, it is collectively referred to as blue light in most current research.
Similar to the effects of visible light referenced above, the effects of blue light irradiation in skin cells includes the formation of ROS, DNA damage, loss of cell viability, activation of inflammation pathways and decreases in cell function. Among these, the main danger is the formation of ROS, as the other adverse effects of blue light are most likely a consequence of ROS. Multiple forms of ROS have been identified as forming in cultured cells in response to blue light irradiation, including: H2O2, superoxide, singlet oxygen and hydroxyl radicals,5, 6 although the induction of singlet oxygen has recently been questioned.7
One of the main intracellular organelles thought to be a target for blue light, and responsible for the formation of these ROS, is the mitochondria.5 In addition, blue light can be absorbed by other types of intracellular molecules such as: flavins; porphyrins; carbonylated proteins—formed when basic residues in proteins react with the aldehydes formed during lipid peroxidation; nitrosated proteins—formed when sulfur containing side chains in proteins react with NO; and lipofuscin.8, 2, 6
When blue light is absorbed by the mitochondria or any of these intracellular molecules, this can result in the formation of ROS, which in turn can damage the intracellular components of the cell leading to a loss of cellular function or even to cell death. Thus, from a skin care perspective, there is intense interest in finding new materials to prevent the adverse effects of blue light irradiation.
It is important to make sure that the actives found to protect against blue light do not interfere with its beneficial aspects.
Screening for Blue Light Protection
There are many ways to generate blue light for cell culture studies in a laboratory setting. One way is to equip a solar simulator with filters to remove any light with a wavelength below 400 nm and above 500 nm. However, an easier alternative is to make use of current LED light technologies. Not only are these types of lights commercially available and easy to find, they are designed to emit light in discrete wavelength intervals in the 400–500 nm range.
Keep in mind the intensity of the LEDs being used. Depending on the endpoint of interest, the dose of blue light needed to achieve biological effects can be as little as 1–5 J/cm2 or potentially greater than 200 J/ cm2.9, 10
Once a source of blue light has been established, the next step to screen materials for blue light-induced ROS would be to decide the means of measuring ROS. Since mitochondria are one of the prime targets for blue light, a good place to start would be an ROS indicator specific to mitochondria.
Commercially available fluorescent indicators are readily available, such as mitoSOX. Besides preferentially accumulating in the mitochondria, mitoSOX also selectively interacts with one of the main ROS species formed by blue light: superoxide. Alternatively, mitochondrial ROS formation can be measured using MitoPY1. Again, this indicator preferentially accumulates in the mitochondria; however it is specific for H2O2, which is another ROS generated by blue light irradiation.
With respect to both mitochondrial ROS indicators, not only can fluorescence intensity be quantitatively measured using a fluorometer, to determine if an active is effective at reducing ROS, but they also can be visualized with fluorescence microscopy for presentation purposes.
The sensitivity of mitochondria to blue light irradiation cannot be understated. Since they are more susceptible to blue light damage than the cell nucleus, the DNA damage associated with blue light-induced ROS production appears to occur preferentially in mitochondrial DNA rather than in genomic DNA.6 In addition, the adverse effects of blue light irradiation on cell function or cell viability seems to be linked to alterations in mitochondrial metabolism.10
There are ways to specifically measure mitochondrial DNA damage using quantitative PCR,11 as well as fluorescent dye indicators that react to mitochondrial functional ability (JC-1, mitochondrial membrane potential dye). While the main importance of an active would be to reduce mitochondrial blue light-induced ROS, if an active could also be shown to reduce mitochondrial DNA damage and/or to maintain mitochondrial function, then that would be extra support for protection against blue light-induced cellular damage.
It may not be necessary to show that an active ingredient specifically reduces ROS formation within the mitochondria, but rather that it reduces ROS content within the entire cell. Intracellular ROS indicators have been around for a while and include nonspecific ROS indicators such as 2',7'-dichlorodihydrofluorescein diacetate (DCDF), or ROS species specific indicators such as dihydroethidium (DHE, specific for superoxide), peroxyfluor-1 (PF1, specific for H2O2) and hydroxyphenyl fluorescein (HPF, specific for hydroxyl radical and peroxynitrite). It should be noted that materials with antioxidant properties have been shown to not only successfully reduce blue light-induced intracellular ROS formation, but also preserve normal cellular function.12
Future Perspective
As dangerous as blue light irradiation is to the skin, there is new and emerging research that indicates all of the cells in the skin (keratinocytes, fibroblasts, melanocytes and hair follicle cells) express photoreceptor proteins designed to be activated by exposure to blue light. These photoreceptor proteins are members of the opsin family—the same family of proteins that enables our eyes to see—and their function in the skin is only now starting to be understood.
In melanocytes opsin-3 activation via blue light promotes melanin synthesis,13 while in hair follicles blue light activation of opsin-2 and/or opsin-3 has been shown to stimulate hair growth.14 Opsin-4 has even been found in subcutaneous white adipocytes, easily reached by visible light penetrating through the skin, and blue light activation of this photoreceptor has been shown to promote an increase in adipocyte lipolytic rate and a decrease in lipid content.15
These are all skin functions that depend on blue light for activation and are potentially beneficial. It is therefore important to keep in mind that as the search for actives to protect against blue light-induced damage continues, the actives identified should not interfere with the beneficial aspects of blue light. Antioxidant-based actives may have an advantage in this regard, since they would scavenge blue light-induced ROS but would have little impact on blue light/photoreceptor interaction.
References
- MF Holick, Biological effects of sunlight, ultraviolet radiation, visible light, infrared radiation and vitamin D for health, Anticancer Research 36 1345–1356 (2016)
- ZCF Garza, M Born, PAJ Hilbers, NAW van Riel, J Liebmann, Visible light therapy: Molecular mechanisms and therapeutic opportunities, Current Medicinal Chemistry [Epub ahead of print] (2017)
- BH Mahmoud, CL Hexsel, IH Hamzavi, HW Lim, Effects of Visible Light on the Skin, Photochemistry and Photobiology 84 450–462 (2008)
- F Liebel, S Kaur, E Ruvolo, N Kollias, MD Southall, Irradiation of skin with visible light induces reactive oxygen species and matrix-degrading enzymes, J Inves Derm 132 1901–1907 (2012)
- PR Hockberger, TA Skimina, VE Centonze, C Lavin, S Chu, S Dadras, J Reddy, J White, Activation of flavin-containing oxidases underlies light-induced production of H2O2 in mammalian cells, Proceedings of the National Academy of Science USA 96 6255–6260 (1999)
- BF Godley, FA Shamsi, F Liang, SG Jarrett, S Davies, M Boulton, Blue light induces mitochondrial DNA damage and free radical production in epithelial cells, J Biological Chem 280(22) 21061–21066 (2005)
- Y Nakashima, S Ohta, AM Wolf, Blue light-induced oxidative stress in live skin, Free Radical Biology and Medicine 108 300–310 (2017)
- T Mizutani, H Sumida, Y Sagawa, Y Okano, H Masaki, Carbonylated proteins exposed to UVA and blue light generate reactive oxygen species through a type I photosensitizing reaction, J Dermatological Science 84(3) 314–321 (2016)
- A Mamalis, M Garcha, J Jagdeo, Light emitting diode-generate blue light modulates fibrosis characteristics: Fibroblast proliferation, migration speed and reactive oxygen species generation, Lasers in Surgery and Medicine 47 210–215 (2015)
- DS Masson-Meyers, VV Bumah, CS Enwemeka, A comparison of four methods for determining viability in human dermal fibroblasts irradiated with blue light, J Pharmacol and Toxicol Methods 79 15–22 (2016)
- A Furda, JH Santos, JN Meyer, B Van Houten, Quantitative PCR-based measurement of nuclear and mitochondrial DNA damage and repair in mammalian cells, Methods in Molecular Biology 1105 419–437 (2014)
- A Mamalis, E Koo, J Jagdeo, Resveratrol prevents reactive oxygen species-induced effects of light emitting diode-generated blue light in human skin fibroblasts, Dermatologic Surgery 42(6) 727-732 (2016)
- C Regazzetti, L Sorman, D Debayle, F Bernerd, MK Tulic, GM De Donatis, B Chignon-Sicard, S Rocchi, T Passeron, Melanocytes dense blue light and regulate pigmentation through opsin-3, J Inves Dermatol [Epub ahead of print] (2017)
- S Buscone, AN Mardaryev, B Raafs, JW Bikker, C Sticht, N Gretz, N Farjo, NE Uzunbajakava, NV Botchkareva, A new path in defining light parameters for hair growth: Discovery and modulation of photoreceptors in human hair follicle, Lasers in Surgery and Medicine 49(7) 705–718 (2017)
- K Ondrusova, M Fatehi, A Barr, Z Czarnecka, W Long, K Suzuki, S Cambell, K Philippaert, M Hubert, E Tredget, P Kwan, N Touret, M Wabitsch, KY Lee, PE Light, Subcutaneous white adipocytes express a light sensitive signaling pathway mediated via a melanopsin/TRPC channel axis, Scientific Reports 7 1–9 (2017)