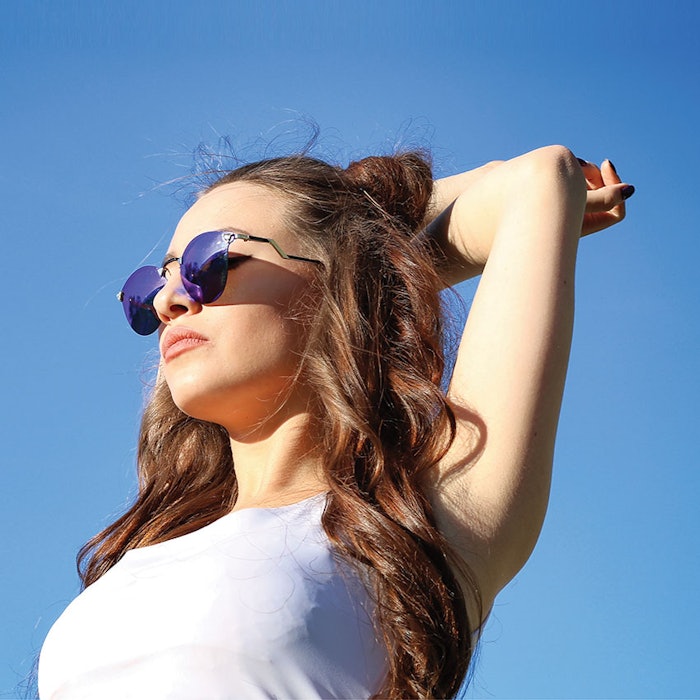
Blue light, or high energy visible (HEV) light, is usually defined as light emitted by the sun in wavelengths ranging from 400–500 nm.1 Approximately 40% of the solar radiation reaching the earth’s surface is infrared light (> 800 nm), while 55% is visible light (400–800 nm) and ~7% is UV light (290–400 nm).2
Log in to view the full article
Blue light, or high energy visible (HEV) light, is usually defined as light emitted by the sun in wavelengths ranging from 400–500 nm.1 Approximately 40% of the solar radiation reaching the earth’s surface is infrared light (> 800 nm), while 55% is visible light (400–800 nm) and ~7% is UV light (290–400 nm).2
The damaging effects of UV on skin are well-studied and documented, as recent reviews illustrate.3–6 In particular, UV irradiation is considered the main contributor to skin photoaging, leading to fine lines, sagging and wrinkles, as well as thinning of the skin, hyperpigmentation and age spots. At a molecular level, these events are caused by: extracellular matrix degradation in the dermis, mainly of the collagen and elastic fibers; decreased cellular proliferation; and the disrupted regulation of pathways related to melanogenesis. These major cellular events result in DNA damage, inflammation, oxidative stress and apoptosis.
Also, over-exposure to UV irradiation is still believed to be the main cause behind various types of skin disorders with decreased barrier function.7, 8 These can range from dry and sensitive skin, to actinic keratosis and malignant skin cancers.4, 9 On the other hand, little is as yet known about the effects of visible light—specifically, blue light—on the skin, which is just adjacent to the UVA region.
Blue light is already known as a therapeutic treatment for cutaneous disorders such as eczema,10 psoriasis11 and acne-like conditions.12, 13 In combination with photosensitizers such as 5-aminolevulinic acid, blue light is also used to treat actinic keratosis.14, 15 In this context, short-term blue light irradiation at a low dose has been shown to have no detectable negative impact on skin in vivo, and has been presumed to be safe.16 However, there is a distinct lack of human studies with larger subject panels investigating long-term exposure or accumulation of blue light doses over weeks or months.
Blue Light in the Literature
What evidence is there for blue light-induced damage? Interestingly, Opländer et al. found that visible light at wavelengths of 410 nm and 420 nm led to cytotoxicity in human dermal fibroblasts, while visible light at 453 nm and 480 nm did not.17 They speculated this was due mainly to the higher energy of wavelengths closer to UV light. Other studies indicated that blue light evokes similar effects to those of UV light, and therefore protection against solar radiation should also include wavelengths above 400 nm.1, 18
A good literature basis can be found for blue light-induced skin damage via the formation of reactive oxygen species (ROS).19–22 This shows conventional sunscreens dedicated to absorbing only UVB and UVA are not able to prevent blue light-induced oxidative stress in skin—only by adding antioxidants such as tocopherol was the formation of ROS blocked.19
Liebel et al. showed that blue light induced the expression of MMP-1 and the pro-inflammatory chemokine IL-8. However, there was no formation of pyrimidine dimers, as seen with UV light.19 Therefore, it seems that blue light contributes primarily to photoaging and inflammation.
Skin has developed defense systems against oxidative stress, including the enzymes catalase, superoxide dismutase and glutathione peroxidase,23 which reduce the adverse effects of UV and visible light irradiation in skin.24 In addition, there are cutaneous antioxidants that cannot be synthesized by skin itself, such as vitamins C and E and carotenoids.25
However, these degrade rapidly upon oxidative stress and must be replenished. Indeed, using Raman spectroscopy, Vandersee et al. showed a significant decrease in cutaneous carotenoids (21%) directly after blue light irradiation at 100 J/cm2.26 In relation, Herrling et al. stated that for complete protection, antioxidative substances must be added to sun filters.28
These findings suggest that to achieve full protection against sun-induced skin aging, sun protection must extend beyond UV light into the visible spectrum.27 Although so far, only titanium dioxide (TiO2), zinc oxide (ZnO) and methylene bis-benzotriazolyl tetramethylbutylphenol (MBBT) have shown absorbance spectra reaching into visible light; and the protection they provide is reportedly low.19, 29, 30
Consumers are gradually becoming aware of blue light risks, but of the 40,000 skin care products launched in 2016, just nine claimed blue light protection.
-Global Cosmetic Industry
When it comes to pigmentation pathways, visible light can also induce hyperpigmentation in vivo,31–34 presumably by inducing the melanogenesis rate-limiting enzyme tyrosinase, as was shown ex vivo.31 This suggests that visible light contributes to the formation of uneven skin tone or solar lentigines. And due to blue light’s longer wavelength, compared with UV, it penetrates more deeply into the skin—down to the subcutis1—but the damaging effects caused in these tissues are currently unknown.
Furthermore, blue light emission stems from solar irradiation as well as electronic devices such as computer screens, tablets and smartphones. Blue light is particularly present in light-emitting diodes (LEDs), too. There is evidence that this wavelength of light has adverse effects on the eyes, including potential retinal damage and digital eye strain.35, 36
With respect to skin, blue light emitted from LEDs of 412–426 nm was shown to lead to the apoptosis of keratinocytes in vitro.37 Another study found that irradiation of dermal fibroblasts with blue light (415 nm and 350 J/cm2) from an LED source inhibited proliferation and migration speed, and led to the generation of ROS.38 These studies suggest blue light irradiation from LED-emitting devices may indeed have damaging effects on skin, contributing to photoaging.
Taken together, this paper investigates the damage caused by blue light using a convenient in vitro test model based on β-carotene. The potential of UV filters to provide protection is assessed, as is the possibility for specific vitamins to complete the array of protection. Further, a model of blue light-induced protein damage was established ex vivo with a new marker of protein carbonylation.
Results show how vitamins, in addition to an extract from the fresh water microalga Scenedesmus rubescens, suppress blue light damage in skin. S. rubescens was chosen as it has previously been found to protect cells from UV irradiation in vitro (data not shown). It is also relatively easy to grow under laboratory conditions and yields a high biomass.
In vitro Materials and Methods
Test compounds: The specific test compounds used in this study, including sunscreens, vitamins and an algae extract, are detailed in Table 1.
β-carotene test: The β-carotene test (BCT) was performed as described previously.39 Due to the light sensitivity of β-carotene, the test was performed in a laboratory equipped with red light. Using a glass syringe, 2 μLcm-2 of β-carotene (0.5% w/w) dissolved in o-xylene and applied to PMMA plates sandblasted to a 2-μm roughness. The plates were then dried in the dark at room temperature for 10 min, to which 2 μLcm-2 of the test formulations were applied as a second layer and homogenously distributed by technicians using their fingertips.
Full spectrum irradiation: The test formulas used were standard o/w emulsions based on the emulsifier potassium cetyl phosphate (see Table 2). The plates were fixated in a xenon testera and irradiated in the range of 290–800 nm with a dose of 329 kJm-2. In the next step, both the cream and β-carotene were dissolved off the PMMA plates in 50 mL of isopropanol, then placed in an ultrasonic bath for 1 min. The blue light range absorption maximum of the β-carotene was photometrically quantified at 452 nm.
Measurements and calculations: For each formulation, three plates were prepared and their average values were calculated. Due to the instability of β-carotene in presence of oxygen, the tests were performed quite quickly. Indeed, such kinetics only allowed for four formulas to be tested at once in order to avoid inconsistencies due to auto-oxidation. Consequently, in one test set-up, the base formulation with and without irradiation was always measured, together with a maximum of two sample formulations. This, in the results shown, two test set-ups combining six data measurements for the base formulation and three data measurements for the sample formulations are depicted.
After calculating averages, the percent of degradation of the irradiated β-carotene was calculated for the base formulation (see formula A in Table 2) and compared with the non-irradiated β-carotene base formulation. The irradiated base formula showed significant degradation of β-carotene, although not completely to zero. In order to compare results from the different test set-ups, the degradation of β-carotene in the base formulation was set to 100% in each test set-up and the protection values of samples were calculated in relation to that. Thus, the irradiated base formulation was normalized as base level protection and the test samples were calculated relative to this value as added protection.
Effects of vitamins: In a second test, the effect of different vitamins was determined in a formulation containing UVA and UVB filters and providing an SPF 50 (see Table 3). Following the test procedure described, β-carotene was again photometrically quantified at 452 nm. The calculations were also performed in the same way, and again the degradation of β-carotene in the base formulation—in this case, formula E (see Table 3)—was set to 100% and the protection values of all samples were calculated in relation to that.
Visible light irradiation: A third test was also performed based on the same formulas from the second test but this time, UV light cut-off filters were appliedb. These filters are dense for wavelengths below 400 nm, thus only wavelengths of 400–800 nm reached the samples. Here, the irradiation dose was 246 kJm-2 and the measurements and calculations were performed in the same manner as described.
Skin Viability
To assess skin viability, two skin punches for each of the selected times, described next, were weighed and their dermal portion reduced as necessary to obtain samples of approximately the same weight. The samples were washed and processed using methylthiazolyldiphenyl-tetrazolium bromide (MTT), which converts to water-insoluble, dark-blue colored MTT formazan by mitochondrial dehydrogenases. The blue crystals were then solubilized and the color intensity, which is directly proportional to skin vitality, was measured with a plate reader at a wavelength of 570 nm. Three readings were taken with each test sample for both punches.
Blue Light-induced ROS, Ex vivo
To detect blue-light induced ROS ex vivo, skin biopsies from abdominal plastic surgery were collected from female donors of the ages 38 and 65, following Helsinki declaration guidelines and with the patients’ informed consent. The tissue from the 38-year-old donor was used to establish ROS detection after blue light irradiation. The tissue from the 65-year-old donor was used to investigate the protective activity of specific compounds.
As a detection probe 2',7'-dichlorodihydrofluorescein diacetate (DCFH-DA) was incorporated into the culture medium for 30 min before irradiation, following the method described by Marionnet et al,40 which the authors adapted for blue light. Compounds were administered topically to the skin tissue before and immediately after blue light irradiation at the concentrations indicated in Figure 1. After changing the medium, blue light was applied at different dosages; i.e., 10 J/cm2, 50 J/cm2 and 100 J/cm2 irradiationc (380–470 nm; max at 420 nm).
A radiometerd equipped with a blue light probee was used to monitor blue light irradiation. Twenty-four hours after irradiation, the skin samples were harvested, cryo-fixed and cut by cryostat for subsequent image acquisition and analysis. For each image, the upper dermis was analyzed by evaluating fluorescence using softwaref. The value obtained was then normalized against the dimension of the selected area.
Literature findings suggest full protection against sun-induced aging requires extending into the visible wavelengths.
Blue Light-induced Protein Carbonylation, Ex vivo
Again, abdominal plastic surgery skin biopsies from female donors, ages 38 and 65, were used to assess protein carbonylation. Tissue from the 38-year-old was used to detect protein carbonylation after blue light irradiation, and tissue from the 65-year-old was used investigate the protective activity of specific compounds.
Compounds were administered topically to skin tissue at the concentrations indicated in Figure 2. After administering compounds, before and immediately following blue light irradiation, carbonylated proteins were extracted from the epidermal part of the skin tissues, as well as 48 hr after irradiationc with blue light (380–470 nm; max at 420 nm).
Once again, a radiometerd equipped with a blue light probee was used to monitor blue light irradiation. After harvesting, the frozen skin samples were weighed and minced. The skin samples for each tested condition were pooled and processed using homogenization in cold extraction buffer. Total cell lysates were then recovered by centrifugation.
Carbonylated proteins in skin samples were determined by Western blot and chemiluminescence detection using a kitg according to the manufacturer’s instructions. The range of the carbonyl content obtained for each sample was normalized against the actin protein signal band.
Statistical Analysis
For ex vivo results, all quantitative data was summarized in terms of the mean score for each treatment and applied measures of variation including standard deviation and standard error of mean (SEM) to the original scores. The student’s t-test was used for unpaired samples.
Results: Full Spectrum β-carotene Degradation
As noted, a previously established BCT was used to assess protection against β-carotene degradation by full-spectrum solar irradiationa. When base formula A (see Table 2) was applied to the β-carotene layer and irradiated with 329 kJm-2 of full-spectrum, solar simulated light, the β-carotene was degraded on average down to 29%. As stated, to compare results from different test set-ups, this degradation was then set to 100% and the protection values of all samples were calculated in relation to it (see Figure 3).
Titanium dioxide, a mineral UV filter capable of absorbing and scattering light, when used at 3% in the formulation, significantly protected β-carotene—maintaining 51%. MBBT, a broad-spectrum UV filter widely used in sunscreens, when used at 4% active substance in the formulation, maintained 63% of β-carotene. The combination of 3% titanium dioxide and 4% MBBT, however, increased the protection level of β-carotene to 86%. The ability to protect β-carotene at 452 nm suggests that both titanium dioxide and MBBT could effectively shield skin against not only UV light, but also against blue light.
In contrast, when oil-soluble UVB or UVA filters are added to the formulation, e.g., bis-ethylhexyloxyphenol methoxyphenyl triazine, ethylhexyl methoxycinnamate, octocrylene, polysilicone-15 or butyl methoxydibenzoyl methane, no protection of β-carotene was detected (data not shown). This confirmed the absorbance and scattering activity of titanium dioxide and MBBT above 400 nm is essential for the protection of β-carotene.
Results: Vitamins vs. β-carotene Degradation
To determine whether the protection afforded by UV filters shown in Figure 3 could be expanded upon, the authors hypothesized gaps in protection might occur from ROS induced either by UV light not fully absorbed by the UV filters, or by wavelengths generating ROS above the main protection spectrum of titanium dioxide and MBBT. Therefore, vitamins were added to a formulation containing different oil-soluble UVB and UVA filters, as well as titanium dioxide and MBBT to ultimately reach an SPF 50.
When the base formula (formula E in Table 3) was applied to the layer of β-carotene and irradiated with 329 kJm-2 of full-spectrum solar-simulated light (290-800 nm), the β-carotene was degraded, on average, down to 60%. Once again, in order to ensure results from different test set-ups were comparable, the degradation of base formula E was set to 100% and the protection values provided by test samples were calculated in relation to that (Figure 4).
Adding 3% niacinamide (vitamin B3) enhanced β-carotene protection to 23% (see Figure 4). Combining 3% niacinamide (vitamin B3) with 0.5% dl-α-tocopherol (vitamin E) in the formula increased protection to 63%.
The improved stability of the β-carotene is attributable to the antioxidant capacities of niacinamide and dl-α-tocopherol. Since the formulation base already contained a UV filter combination to achieve an SPF of 50 (see Table 3), which absorbed a significant amount of UVB and UVA radiation, it could be concluded that the vitamins provided added protection against the adverse effects of oxidative stress caused by visible light.
Results: Blue Light β-carotene Degradation
Since the absorbance maximum of β-carotene falls at the blue light wavelength of 452 nm, the BCT is particularly useful to assess blue light damage. Thus, as a next step, the authors sought to confirm the hypothesis that the additional β-carotene protection by vitamins B3 and E was due to the quenching of oxidative stress evoked by blue light; note that vitamin B6 was also included in this advanced test set-up. For this purpose, the UV light spectrum was blocked using specialized filters. This way, only wavelengths above 400 nm were irradiated.
Again, the SPF 50 base formulation (formula E in Table 3) was applied on top of the β-carotene layer and irradiated with a dose of 246 kJm-2 within the 400–800 nm spectrum. Under these conditions, β-carotene was degraded, on average, down to 64%. As noted, the degradation of the base formula (formula E) was again set to 100% so that each test set-up and its results were comparable (see Figure 5).
Interestingly, adding 1% pyridoxine hydrochloride (vitamin B6) enhanced β-carotene protection by 19% (see Figure 5) and the addition of 3% niacinamide (vitamin B3) enhanced β-carotene protection by 22%. However, when both pyridoxine hydrochloride and niacinamide (vitamins B6 and B3) were combined, protection strongly increased to 50%. Since this protective effect was greater than the sum of the two individual vitamin results, a synergistic effect was identified.
Further, the combination of niacinamide (vitamin B3) and 0.5% dl-α-tocopherol (vitamin E) provided β-carotene protection at 65%. Notably, vitamins B3, E and the B6/B3 combination provided nearly the same results as in Figure 4, with full spectrum irradiation. Here, the formulas contained an effective mixture of UV filters to shield against UVB and UVA but this did not provide 100% protection, and some photons could still reach the skin.
As noted, to exclude any UV radiation from this test, an additional UV cut-off filter was used. Yet, even excluding UV light, significant protection was provided by the tested vitamins. A similar result with both full-spectrum and visible light confirmed the vitamins have the greatest impact above 400 nm. And since this method used the absorbance maximum of β-carotene at 452 nm, it also shows that formulations containing 1% pyridoxine hydrochloride (vitamin B6), 3% niacinamide (vitamin B3), 0.5% dl-α-tocopherol (vitamin E) and combinations thereof can protect β-carotene against the harmful effects of blue light.
Results: Blue Light vs. Skin Viability, ROS and Protein Carbonylation
As described, to establish a skin model for blue light irradiation, skin tissue from a 38-year-old female donor was exposed to blue light (380–470 nm) and assessed for oxidative stress by examining ROS formation19 and protein carbonylation. ROS formation was previously shown to increase markedly by visible and blue light.19, 20 Therefore, the authors used this marker as a positive control to set up the model.
As shown in Figure 6a, ROS formation increased significantly and dose-dependently upon irradiation with 10 J/cm2, 50 J/cm2 and 100 J/cm2 blue light (+6,088%; p < 0.01 vs. control). Protein carbonylation can be caused by oxidative stress and induced by UV irradiation.41 And since most in vitro skin damage found thus far resembled damage induced by UV irradiation, the authors speculated that protein carbonylation could also result from blue light irradiation.
In Figure 6b, protein carbonylation was indeed shown to increase significantly and dose-dependently (+40%; p < 0.05 vs. control at 100 J/cm2 blue light). This confirmed the hypothesis.
Concerning cytotoxic effects, an MTT assay42 was performed to assess tissue viability after irradiation with 100 J/cm2 blue light; no cytotoxic effect was observed. This suggested irradiation took place at tolerable fluencies (see Figure 7).
Test Model in Practice
Blue light-induced ROS vs. test compounds: Having established an ex vivo blue light irradiation model, the authors next tested the protective activity of vitamins B3 and B6, and S. rubescens extract. Skin tissue from the 65-year-old female donor was exposed to 100 J/ cm2 blue light (380-470 nm) in the presence of these compounds (see Figure 1). A significant reduction in blue light-induced ROS in skin ex vivo was observed in the presence of 3% niacinamide (vitamin B3) (-48%; p < 0.01 vs. irradiated control) and 0.075% S. rubescens dry extract (-35%, p < 0.05) (see Figure 1). No significant activity was observed for vitamin B6 (see Figure 1) and none of the treatments exhibited cytotoxic effects when combined with blue light irradiation, as assessed by the MTT assay (data not shown).
Blue light-induced carbonylation vs. test compounds: Next, the compounds used in Figure 1 were tested for their ability to suppress protein carbonylation induced by blue light irradiation. Again, tissue from the 65-year-old donor was irradiated with 100 J/cm2 blue light (380–470 nm). When normalized to the non-irradiated vehicle control, a significant 93% reduction in protein carbonylation was observed with 3% niacinamide (vitamin B3) (p < 0.05 vs. irradiated control). With 0.25% and 0.75% S. rubescens dry extract, a significant 85% reduction (p < 0.05 vs. irradiated control) was observed (see Figure 2). Vitamin B6 also showed a tendency to inhibit protein carbonylation (see Figure 2).
The addition of vitamins increased protection against oxidative stress specifically caused by visible light.
Discussion
The damaging effects of UV irradiation on skin have been reported extensively, and its role in cutaneous carcinogenesis and photoaging is well-accepted.5, 43, 44 More recently, the possible damaging effects of visible light have also come into focus.1, 45 As such, the described BCT is an effective in vitro test to screen for substances that are capable of protecting against or quenching blue light irradiation-induced ROS.39
β-Carotene is a particularly blue light-sensitive molecule that rapidly quenches ROS, such as singlet oxygen and peroxides. Carotenoids are well-described antioxidants that are present in many foods and plants, and protect skin from light-induced damage.46 In addition, they have light-absorbance maxima in the visible range, mainly in the blue light spectrum, and could be considered biological protectants against solar irradiation via oral intake.47
Accumulating evidence suggests protection from solar irradiation-induced cutaneous damage must go beyond UV irradiation,1, 27, 31 as UV filters usually protect against wavelengths below 400 nm only. Few UV filters, e.g., titanium dioxide, MBBT and ZnO, have absorption spectra into the visible range.18, 29 In this respect, Liebel et al. showed the visible light induction of ROS could only be reduced significantly if antioxidants such as gamma-tocopherol were present alongside UVA/UVB-absorbing sun filters.19
In the present work, 3% titanium dioxide and 4% MBBT (active substance) in a formulation were able to block 50% of blue light transmission between 400 nm and 500 nm (data not shown). Surprisingly, using the BCT test, a formulation containing the two substances resulted in an 86% reduction in β-carotene degradation (see Figure 3). Obviously, shielding light at wavelengths above 400 nm is important to protect skin effectively not only against the degradation of β-carotene—a significant antioxidant in the skin—but also for its apparent benefits against oxidative stress.
Adding niacinamide (vitamin B3) to the formula, which has previously been found to exhibit antioxidant and even DNA repair capacity,48, 49 provided further benefits with regard to solar irradiation-induced ROS β-carotene degradation. In addition, vitamin E, in this case dl-α-tocopherol, improved protection further, again confirming the previous findings that vitamin E (gamma-tocopherol) improves protection against solar light-induced skin damage19 (see Figure 4). By blocking UV light below 400 nm, the authors also showed that the vitamin-dependent increase in protection against oxidative stress was visible light-specific (see Figure 5).
The results obtained with visible light-irradiated formulas resembled those exposed to full-spectrum irradiation, confirming the vitamins had their main impact above 400 nm. Especially good results were obtained with combinations of niacinamide (vitamin B3) and pyridoxine hydrochloride (vitamin B6), as well as with niacinamide (vitamin B3) and dl-α-tocopherol (vitamin E). These results further corroborate the usefulness of the BCT as a screening tool for antioxidative agents the reach beyond UV. They also underline the need for additional cutaneous protection that encompasses the visible light spectrum.
To show the relevance of BCT test findings in skin, an ex vivo model of blue light skin damage was developed. As previously established, increased oxidative stress was observed when measuring ROS with the DCFH-DA fluorescent probe (see Figure 6a). Samples were irradiated using a devicec that has a wavelength range of 380–470 nm, with a peak at 420 nm, which is therefore focused on the lower wavelengths of the blue light spectrum. Devices with similar wavelengths were used previously by others to study visible or blue light-dependent skin damage.16 Lewis et al., for example, used a QTH light chamber with a 380–500 nm wavelength.20 The fluencies used, of up to 100 J/cm2 blue light, were in the ranges of those reported by others. Using a digital phototherapy deviceh, for example, Vandersee et al. showed that maximum β-carotene destruction in vivo happened at blue light fluencies (380–495 nm) of 100 J/cm2.26 Regarding the relevance of such a dose, Hockberger et al. stated that blue light at irradiation doses of 2–6 J/cm2 is equivalent to 5–10 min of direct sunlight,21 meaning that 100 J/cm2 is approx. 250–500 min.
One marker of cutaneous oxidative stress previously not investigated in the context of visible or blue light irradiation is protein carbonylation. It has, however, been shown to result from UV irradiation.41 Protein carbonylation is the addition of reactive carbonyl groups, such as aldehydes and ketones, to proteins due to oxidative stress, which leads to protein oxidation.50, 51 The finding that blue light can cause an increase in protein carbonylation ex vivo (see Figure 6b), presented here, is in line with the finding that blue light causes an increase in ROS ex vivo (see Figure 6a). Interestingly, another very recent study found that carbonylated protein exposed to blue light itself generates ROS.52
Together with the results presented here, this may suggest the formation of ROS and protein carbonylation represents a kind of blue light-induced vicious cycle of oxidative damage in skin. Protein carbonylation and the glycation of proteins correlate with an age-dependent increase in yellowish skin tone and skin tone heterogenicity, as was shown in Japanese subjects,53, 54 and this provides further evidence that blue light contributes to facial skin aging—and that protection from blue light irradiation helps prevent accelerated photoaging.
While the results shown in Figure 2 do not explain how vitamins prevented blue light-induced protein carbonylation, the authors hypothesize they quench ROS and therefore suppress the downstream formation of protein oxidation, since anti-oxidative potential was shown for all the vitamins tested.25, 48, 55 Niacinamide and the S. rubescens extract significantly inhibited both ROS and protein carbonylation (see Figure 1 and Figure 2).
Finally, it is worth noting that vitamin B6 showed a tendency toward the inhibition of carbonylated proteins but not ROS. It could be speculated that this may involve alternative mechanisms, such as the activation of additional anti-oxidative enzymes, instead of direct ROS quenching. Nevertheless, all three vitamins seemed to act in some way against blue light-induced oxidative stress in ex vivo skin samples.
Conclusions
In summary, this data provides further evidence that visible light, particularly blue light, can promote harmful effects such as oxidative damage in skin. It can do so by decreasing the skin’s own protective mechanisms, including β-carotene content. Protection beyond the UV spectrum of solar irradiation should therefore become paramount to protecting the skin against sun-induced aging. As a result of this study, the authors propose a three-dimensional protection strategy for skin using: UV filters that reduce the transmission of blue light in the range of 400 nm to 500 nm; vitamins such as B3, B6 and E; as well as unique extracts such as S. rubescens to defend skin on different levels against damaging irradiation.
Acknowledgements: The authors wish to thank their colleagues, Thomas Rudolph, Anne Janssen, Jochen Klock, Juergen Vollhardt, Mathias Gempeler and Aline Huber, for their helpful comments.
References
- E Dupont, J Gomez and D Bilodeau, Beyond UV radiation: A skin under challenge, Int J Cosmet Sci 35 224-232 (2013)
- OV Dueva-Koganov et al, In vitro evaluation of potential protection provided by topical products against full solar and visible plus infrared radiation, Household and Personal Care Today 9(2) 37-43 (2014)
- H Chen, QY Weng and DE Fisher, UV signaling pathways within the skin, J Invest Dermatol 134(8) 2080-5 (2014)
- J D'Orazio et al, UV radiation and the skin, Int J Mol Sci 14(6) 12222-48 (2013)
- RE Watson et al, Damage to skin extracellular matrix induced by UV exposure, Antioxid Redox Signal 21(7) 1063-77 (2014)
- C Battie et al, New insights in photoaging, UVA induced damage and skin types, Exp Dermatol 23 suppl 1, 7-12 (2014)
- K Biniek, K Levi and RH Dauskardt, Solar UV radiation reduces the barrier function of human skin, Proc Natl Acad Sci USA 109(42) 17111-6 (2012)
- R Voegeli et al, Increased basal transepidermal water loss leads to elevation of some but not all stratum corneum serine proteases, Int J Cosmet Sci 30 435–442 (2008)
- FR de Gruijl, Skin cancer and solar UV radiation, Eur J Cancer 35(14) 2003-9 (1999)
- K Keemss et al, Prospective, randomized study on the efficacy and safety of local UV-free blue light treatment of eczema, Dermatology 232(4) 496-502 (2016)
- S Pfaff et al, Prospective randomized long-term study on the efficacy and safety of UV-free blue light for treating mild psoriasis vulgaris, Dermatology 231(1) 24-34 (2015)
- S Ammad et al, An assessment of the efficacy of blue light phototherapy in the treatment of acne vulgaris, J Cosmet Dermatol 7(3) 180-8 (2008)
- C Antoniou et al, A multicenter, randomized, split-face clinical trial evaluating the efficacy and safety of chromophore gel-assisted blue light phototherapy for the treatment of acne, Int J Dermatol 55(12) 1321-1328 (2016)
- DJ Piacquadio et al, Photodynamic therapy with aminolevulinic acid topical solution and visible blue light in the treatment of multiple actinic keratoses of the face and scalp: Investigator-blinded, phase 3, multicenter trials, Arch Dermatol 140(1) 41-6 (2004)
- J Gandy et al, Photodynamic therapy effectively treats actinic keratoses without pre-illumination incubation time, J Drugs Dermatol 16(3) 275-278 (2017)
- 16. MM Kleinpenning et al, Clinical and histological effects of blue light on normal skin, Photodermatology, Photoimmunology & Photomedicine 26 16-21 (2010)
- C Oplander et al, Effects of blue light irradiation on human dermal fibroblasts, J Photochem Photobiol B 103(2) 118-25 (2011)
- BH Mahmoud et al, Effects of visible light on the skin, Photochem Photobiol 84(2) 450-62 (2008)
- F Liebel et al, Irradiation of skin with visible light induces reactive oxygen species and matrix-degrading enzymes, J Invest Dermatol 132 1901-1907 (2012)
- JB Lewis et al, Blue light differentially alters cellular redox properties, J Biomed Mater Res B Appl Biomater 72(2) 223-9 (2005)
- PE Hockberger et al, Activation of flavin-containing oxidases underlies light-induced production of H2O2 in mammalian cells, PNAS 96 6255-6260 (1999)
- Y Nakashima, S Ohta and AM Wolf, Blue light-induced oxidative stress in live skin, Free Radic Biol Med 108 300-310 (2017)
- TW Fischer et al, Melatonin enhances antioxidative enzyme gene expression (CAT, GPx, SOD), prevents their UVR-induced depletion, and protects against the formation of DNA damage (8-hydroxy-2’-deoxyguanosine) in ex vivo human skin, J Pineal Res 54 303-312 (2013)
- J Fuchs et al, Acute effects of near ultraviolet and visible light on the cutaneous antioxidant defense system, Photochem Photobiol 50(6) 739-44 (1989)
- F McArdle et al, Effects of oral vitamin E and beta-carotene supplementation on ultraviolet radiation-induced oxidative stress in human skin, Am J Clin Nutr 80(5) 1270-5 (2004)
- S Vandersee et al, Blue-violet light irradiation dose dependently decreases carotenoids in human skin, which indicates the generation of free radicals, Oxid Med Cell Longev 579675 (2015)
- L Kolbe, How much sun protection is needed? Are we on the way to full-spectrum protection? J Invest Dermatol 132(7) 1756-7 (2012)
- T Herrling and K Jung, The radical status factor (RSF): A novel metric to characterize skin products, Int J Cosmet Sci 34(4) 285-90 (2012)
- N Kollias, The absorption properties of "physical" sunscreens, Arch Dermatol 135(2) 209-10 (1999)
- B Herzog et al, In vivo and in vitro assessment of UVA protection by sunscreen formulations containing either butyl methoxy dibenzoyl methane, methylene bis-benzotriazolyl tetramethylbutylphenol, or microfine ZnO, Int J Cosmet Sci 24(3) 170-85 (2002)
- M Randhawa et al, Visible light induces melanogenesis in human skin through a photoadaptive response, PLoS One 10(6) e0130949 (2015)
- BH Mahmoud et al, Impact of long-wavelength UVA and visible light on melanocompetent skin, J Invest Dermatol 130(8) 2092-7 (2010)
- N Kollias and A Baqer, An experimental study of the changes in pigmentation in human skin in vivo with visible and near infrared light, Photochem Photobiol 39(5) 651-9 (1984)
- MA Pathak, FC Riley and TB Fitzpatrick, Melanogenesis in human skin following exposure to long-wave ultraviolet and visible light, J Invest Dermatol 39 435-43 (1962)
- PV Algvere, J Marshall and S Seregard, Age-related maculopathy and the impact of blue light hazard, Acta Ophthalmol Scand 84(1) 4-15 (2006)
- M Kaido et al, Reducing short-wavelength blue light in dry eye patients with unstable tear film improves performance on tests of visual acuity, PLoS One 11(4) e0152936 (2016)
- J Liebmann, M Born and V Kolb-Bachofen, Blue-light irradiation regulates proliferation and differentiation in human skin cells, J Invest Dermatol 130(1) 259-69 (2010)
- A Mamalis, M Garcha and J Jagdeo, Light emitting diode-generated blue light modulates fibrosis characteristics: fibroblast proliferation, migration speed and reactive oxygen species generation, Lasers Surg Med 47 210-215 (2015)
- T Rudolph et al, State-of-the-art light protection against reactive oxygen species, SOFW J 140(3) 10-14 (2014)
- C Marionnet et al, Diversity of biological effects induced by longwave UVA rays (UVA1) in reconstructed skin, PLoS One 9(8) e105263 (2014)
- CS Sander et al, Photoaging is associated with protein oxidation in human skin in vivo, J Invest Dermatol 118(4) 618-25 (2002)
- T Mosmann, Rapid colorimetric assay for cellular growth and survival: Application to proliferation and cytotoxicity assays, J Immunol Methods 65(1-2) 55-63 (1983)
- DL Narayanan, RN Saladi and JL Fox, Ultraviolet radiation and skin cancer, Int J Dermatol 49(9) 978-86 (2010)
- M Ichihashi et al, UV-induced skin damage, Toxicology 189(1-2) 21-39 (2003)
- D Barolet, F Christiaens and MR Hamblin, Infrared and skin: Friend or foe, J Photochem Photobiol B 155 78-85 (2016)
- E Fernandez-Garcia, Skin protection against UV light by dietary antioxidants, Food Funct 5(9) 1994-2003 (2014)
- W Stahl and H Sies, beta-Carotene and other carotenoids in protection from sunlight, Am J Clin Nutr 96(5) 1179S-84S (2012)
- JP Kamat and TP Devasagayam, Nicotinamide (vitamin B3) as an effective antioxidant against oxidative damage in rat brain mitochondria, Redox Rep 4(4) 179-84 (1999)
- BC Thompson, GM Halliday and DL Damian, Nicotinamide enhances repair of arsenic and ultraviolet radiation-induced DNA damage in HaCaT keratinocytes and ex vivo human skin, PLoS One 10(2) e0117491 (2015)
- M Fedorova, RC Bollineni and R Hoffmann, Protein carbonylation as a major hallmark of oxidative damage: Update of analytical strategies, Mass Spectrom Rev 33(2) 79-97 (2014)
- E Cabiscol, J Tamarit and J Ros, Protein carbonylation: Proteomics, specificity and relevance to aging, Mass Spectrom Rev 33(1) 21-48 (2014)
- T Mizutani et al, Carbonylated proteins exposed to UVA and to blue light generate reactive oxygen species through a type I photosensitizing reaction, J Dermatol Sci 84(3) 314-321 (2016)
- H Ohshima et al, Melanin and facial skin fluorescence as markers of yellowish discoloration with aging, Skin Res Technol 15(4) 496-502 (2009)
- Y Ogura et al, Dermal carbonyl modification is related to the yellowish color change of photo-aged Japanese facial skin, J Dermatol Sci 64(1) 45-52 (2011)
- P Bilski et al, Vitamin B6 (pyridoxine) and its derivatives are efficient singlet oxygen quenchers and potential fungal antioxidants, Photochem Photobiol 71(2) 129-134 (2000)