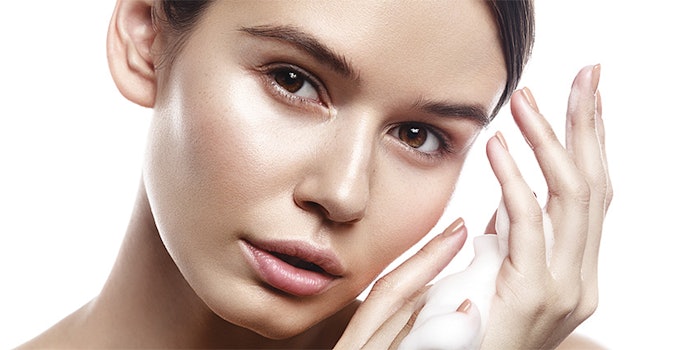
Crosslinked polyelectrolyte thickeners— exemplified by carbomers, acrylate crosspolymers and acryloyldimethyltaurate crosspolymers—confer stable suspension properties, shear-thinning rheology and stimuli-responsive behavior to aqueous formulations. Formulators have fashioned cosmetic compositions around these useful characteristics for more than half a century, but our fundamental understanding of these materials is far from complete—and, amazingly, improvements are continually made to this class of ingredients.
For example: new, stable, dry-powder “latexes” can be processed to impart either “long” or “short” rheology;1 transparent suspending shampoos can be formulated from alkali swellable, acid swellable thickeners;2 or nonionic surfactant-activated microgel thickeners3-5 and superabsorbent polymers can impart emulsion stability and good skin-feel attributes.6 In addition, core-shell microgels based on a cross-linked polyacrylate core and a cationic silicone shella,7 are effective emulsifiers. These enable formulation consistencies, ranging from thin lotions to thick creams, merely by changing the concentration or pH of the emulsions they are stabilizing.
This article reviews the relationship between structure and function for polyelectrolyte crosspolymer rheology modifiers. It also considers their behavior in different systems, for the benefit of formulators.
Chemical Potential vs. Osmotic Pressure
Formulators who have experience with carbomers know that dramatic thickening and the gelation of mucilages occurs when a base or amine is added to a carbomer dispersion. Carbomers essentially consist of cross-linked poly(acrylic acid), and gelation/viscosification occurs when the polymer is neutralized to the corresponding acrylate salt. This increase in viscosity is caused by the osmotic swelling of the resulting polyelectrolyte.8-10
The influence of osmotic pressure can be demonstrated experimentally by placing exemplary materials inside tubular dialysis membranes and hanging them over a large tank of water so they just barely touch the water (see Figure 1). The pores in the membranes are sufficiently large to allow the passage of small molecules such as water, sucrose and small ions such as sodium or chloride. However, polymer molecules are too large to squeeze through the dialysis pores (see Figure 2 and Figure 3).
These experiments illustrate four key scenarios. The first two relate to basic interactions in chemical potential versus osmosis; two additional examples show how osmosis affects materials and systems of increasing size and complexity.
1. Solublility and Chemical Potential
In the first scenario, when ingredients consisting of small, soluble molecules such as sugar or table salt are placed inside the dialysis tubes, the soluble molecules readily diffuse into the reservoir water and the membrane tube “empties” through its pores. This is because the interaction between the ions and water is stronger than the interaction between the sodium and chloride in the crystals. Under these conditions, the chemical potential driving force causes both dissolution and the diffusion of sodium and chloride ions into the bulk water until the concentration of salt is essentially homogeneous throughout the bulk aqueous phase (see Figure 3).
There are limits to the dissolution of salt in water, however. For a saturated solution, the chemical potentials for crystal formation and dissolution become equal. So, if more salt is added to a saturated solution, the chemical potential favors crystal formation over dissolution.
2. Nonionic Polymers and Osmosis
The second scenario is when a small amount, e.g., 1 g, of a nonionic polymer such as hydroxyethylcellulose is placed in the dialysis tube. Here, the water is observed to soak into the dialysis tube and, at equilibrium, the water level in the tube will settle slightly above the level of water in the larger tank. In this case, the water-soluble polymer molecules (the solute) are too large to escape through the pores of the dialysis membrane and, since the polymer cannot diffuse into the water, the water diffuses into the polymer in attempt to form a homogeneous solution (see Figure 3). In this case, the polymer solution is formed by diffusion of the water into the dialysis tube.
Oil droplets and particulates take up space. As such, critical concentrations for polymers in these combined systems will be lower.
The driving force for this process is osmosis. The osmotic pressure driving the water into the dialysis tube is proportional to the molar concentration of solute, and the concentration of water will continue to rise in the tube until the pressure head of water becomes equal to the osmotic pressure. Note that the osmotic pressure arises from the configurational entropy of the chains; but this discussion will be reserved for a future review.
Concentration regimes: It is important to understand the dynamics occurring as system concentrations are formed. As the water diffuses into the polymer solution, three distinct concentration regimes are formed, in sequence: concentrated, semi-dilute and dilute. Knowledge of these three regimes aids the formulator in designing storage-stable compositions that confer desired topical sensory effects. Transitions between these regimes occur at critical polymer concentrations—namely, the critical overlap and critical entanglement concentrations.
Throughout these transitions, the polymer’s molecular shape and size in solution changes, depending upon the nature of the solvent. Polymers can be regarded as “springs” that expand in good solvents and shrink in poor ones. Therefore, the critical concentrations depend upon the nature of the composition in which the polymer is dissolved. Moreover, oil droplets in emulsions and particulates in dispersions also take up space in a composition, leaving less space for the polymers. As a consequence, the critical concentrations for polymers in emulsions and dispersions will be lower than for pure polymer solutions.
Critical overlap and critical entanglement: When a polymer first dissolves in a limited amount of solvent, the polymer concentration will be fairly high and the dissolved polymers’ molecular chains will be entangled with each other. As more solvent diffuses into the solution, the polymer concentration will drop below the critical entanglement concentration, denoted as Ce, and the system will enter the semi-dilute regime. In the semi-dilute regime, the polymer molecules overlap each other but they are no longer entangled. Eventually, with further dilution, the polymer chains will pass the critical overlap concentration, denoted as C*, and enter the dilute regime, in which the polymer molecules each occupy their own exclusive volume in solution and do not overlap with each other.
C* and Ce reiterated: Given the importance of where C* and Ce occur in formulations, it is worth reiterating what these concentrations mean. In the dilute regime, ideally, the polymer molecules are isolated in solution and are free to diffuse, driven by Brownian motion. The upper limit of the dilute regime is C*, the critical overlap concentration. This concentration can be visualized as the concentration at which the polymers just touch sufficiently to reach the percolation threshold of the system.
Above C*, the system enters the semi-dilute regime. At a higher critical concentration, i.e., the critical entanglement concentration or Ce, the system enters the concentrated regime. Ideal polymers in the concentrated regime entangle, and the polymer matrix becomes self-similar throughout the solution.
The locations of C* and Ce are usually determined by plotting the solution-specific viscosity as a function of polymer concentration. The positions of C* and Ce can then be detected as discontinuities in the curve (see Figure 4).11
continue reading in the Digital Edition...
References
- US Pat App 2018/0169445, Stable rheology modifiier compositions, K Rodrigues, A Bailey et al, applied for by Akzo Nobel Chemicals International B.V. (Jun 21, 2018)
- US Pat App 2018/0185263, Clear suspending personal care cleaning compositions, M Fevola, T Fuetterer, J Martin, S Shah and A Zhuk, applied for by Johnson & Johnson Consumer Inc. (Jul 5, 2018)
- US Pat 9,096,755, Surfactant responsive microgels, K Chari, S-J Hsu, W-Y Yang, B Prachur and M Kadir, assigned to Lubrizol Advanced Materials (Aug 4, 2015)
- US Pat App 2016001562, Surfactant responsive microgel polymers and methods to mitigate the loss of silicone deposition from keratinous substrates, D Rafferty, B Figura, W-Y Yang and K Chari, assigned to Lubrizol Advanced Materials (Jan 21, 2016)
- US Pat App 20160304646, Surfactant responsive microgels, S-J Hsu, K Chari and S Li, assigned to Lubrizol Advanced Materials (Oct 20, 2016)
- US Pat 9,839,598, Superabsorbent polymers and sunscreen actives for use in skin care compositions, P Tanner and Manohar, assigned to The Procter & Gamble Company (Dec 12, 2017)
- momentive.com/products/literature/silsoft-eau-microgel-marketing-bulletin/ (accessed Aug 24, 2018)
- PJ Flory, Principles of Polymer Chemistry, Cornell University Press (1953) pp 629-637
- A Katchalsky and Z Alexandrowicz, On the additivity of osmotic properties of polyelectrolyte—Salt solutions, J Polymer Sci A 1(6) 2093 (1963)
- Q Liao, AV Dobrynin and M Rubinstein, Molecular dynamics simulations of polyelectrolyte solutions, osmotic coefficient and counterion condensation, Macromolecules 36 3399 (2003)
- V Padman, Doctoral dissertation, University of Southern Mississippi (2012)